Lab Notes
What Are Free Radicals and Why Should You Care?
Have you been hearing the term Free Radicals a lot in the skincare community and don't know what the fuss is about? Well, we're here to get you up to speed.
What are Free Radicals?
Assuming you’re into skincare, you’ve probably come across the term free radicals many times and read about its association with the sun’s harmful effects on the skin. But what exactly are free radicals and why should a skincare nerd like you and I care about them? A free radical is any atom containing one or more unpaired electrons that is capable of independent existence. An unpaired electron occupies an atomic or molecular orbital by itself and gives free radicals their reactive properties. Electrons are most stable when they exist in pairs so an unpaired electron is always looking for ways to increase its stability. It can do this by stealing electrons from neighboring molecules, creating new free radicals which can then cause various forms of damage. This damage manifests itself on our skin in a variety of ways, the most common being skin aging. An example of a free radical is the hydrogen atom. It has only a single unpaired electron, making it the simplest free radical in existence (Figure 1). Free radicals can react with either other free radicals or other molecules but the reactivity of radicals varys. The rate and selectivity of their reactions are dependent on things like concentration and bond strength.


What are the different sources of Free radicals?
Free radicals are constantly produced in vivo through normal skin processes. Although, at certain levels, mitochondrial ROS production can actually have a number of beneficial effects. ROS/RNS are involved in immune response through the defense of pathogenic microorganisms, and they are also beneficial for cell survival and adaptive signaling (Phaniendra, Jestadi, & Periyasamy, 2015). Our bodies have developed both antioxidant defense systems and repair systems to protect and prevent free-radical damage. Mitochondria play a large role in the production of ROS in vivo. The overproduction of ROS in the mitochondria can damage cells and lead to various diseases and disorders. Oxidative stress is the term that we usually use to describe the overproduction of ROS in the body and its resulting damage. This term has now been split into two terms: oxidative eustress and oxidative destress. Oxidative eustress describes the circumstance when a balance can be formed between ROS production and adaptive cell responses, whereas oxidative distress occurs when the ROS levels have exceeded this point of reversibility and damage is inevitable.
There are many regular skin processes that create an increase in ROS levels in the skin such as during injury, skin repair, and inflammatory diseases. Chronic, non-healing wounds have been found to induce oxidative stress. ROS are produced during the wound-healing process and along with RNS play a role in the regulation of this process. ROS have also been shown to act as secondary messengers in the activation of MAPK/AP1, NF-κB, and JAK/STAT-signaling pathways during the pathogenesis of psoriasis or acne. They also may be involved in the pathogenesis of allergic skin reactions, photodermatosis, and drug eruption. (Xu, H et al., 2017)
Intrinsic source
Most free radicals are produced in the mitochondria as a result of energy production that simulates ROS production via a number of mechanisms. Mitochondrial ROS production occurs through complexes I and III, as well as up to 12 other ROS generators within the mitochondria (Mailloux, 2020). High bursts of succinate during ischemia leads to ROS production. Succinate is oxidized by complex II, leading to the backwards flow of electrons to complex I, producing large amounts of ROS (Mailloux, 2020). These bursts of succinate leads to bursts in ROS production over time through reverse electron transport, which can then lead to free radical-induced damage.

Figure 3: Mitochondrial ROS production via Reverse Electron Transport.
There are several different enzymes within the body that are involved in the production of ROS in vivo. Transmembrane NADPH oxidase can generate superoxide through the transfer of an electron from NADPH to a FAD cofactor, which is then donated to O2 on the extracellular side of the membrane. The proposed mechanism for production of ROS by ɑ-keto acid dehydrogenases also relies on these succinate bursts in complex II. Reverse electron transport drives protons to the matrix by complex I which results in NADH formation (Mailloux, 2020). NADH is then oxidized to NAD+ by the dehydrogenases, leading to ROS formation.

Figure 4: Mitochondrial ROS production by ɑ-keto acid dehydrogenases
Most of the production of superoxide within the body is deliberate although some appears to happen accidently from the leakage of electrons from electron transport chains (Halliwell, 1993). The deliberate production of superoxide mainly comes from activated phagocytes but can also come from different cell types like fibroblast and lymphocytes. SOD enzymes can then convert superoxide into other ROS, O2 and H2O2, which alone do not react directly with DNA but can lead to DNA damage.
Homolytic bond fission can occur in vivo, leading to the production of free radicals. For example, the hydroxyl radical is produced in the body by either the reaction of transition metals with hydrogen peroxide or by the breaking of covalent bonds in water molecules. The fracture and grinding of bone as a result of mechanical damage can also generate free radicals in present collagen due to the breaking of covalent bonds (Halliwell & Gutteridge, 2015).
Extrinsic source
There are also various environmental sources of free radicals including the most commonly know ones, UV radiation, smoking, and air pollution. These factors are well known to contribute to skin aging and various skin disorders. Another interesting source of free radicals is the oil vapours that result from frying during cooking, due to the oxidation that occurs upon exposure to heat. There are various pollutants, such as biomass fuel, that can act as oxidants themselves or act in the production of other reactive oxygen species. This uncontrolled formation of ROS is what can lead to skin disorders such as inflammatory dermatoses. Cigarette smoke is another large contributor of reactive species containing 1017 oxidant molecules per puff (Donohue, 2016).
Exposure to UV radiation is well known to cause skin damage and photoaging which come as a result of increased oxidative stress on the skin due to free radical generation. Ultraviolet light generates ROS which have the ability to alter a cell’s ability to function. When UV radiation touches the skin, it is absorbed by target chromophores, DNA and Urocanic acid. This will lead to photo-induced DNA damage that can result in abnormal gene expression. Urocanic acid, found in the epidermis, will produce the singlet oxygen radical when exposed to UVB. This can then cause damage to the skin and further produce more ROS (Mitchell, 2016). It is known that UVB also stimulates the production of superoxide radicals in fibroblasts but the exact mechanism is unknown. Current theories include the interaction of UV with melanin and the stimulation of the mitochondrial electron transport chain.
What are the different types of Free radicals?
The different types of free radicals can be divided into 3 categories: reactive oxygen species, reactive nitrogen species, and reactive carbonyl species.
Reactive Oxygen Species (ROS)
Reactive oxygen species, the most commonly known group, is a term that includes both radical and non-radical derivatives of oxygen. Non-radicals are more stable than radicals but have the potential to lead to free-radical reactions. The overproduction of ROS is associated with many disorders including skin disorders. However, new evidence suggests that ROS also have a beneficial role within the body, acting as messengers in cellular signaling and participating in the regulation of cell adhesion.
Table 1: Radical and non radical ROS

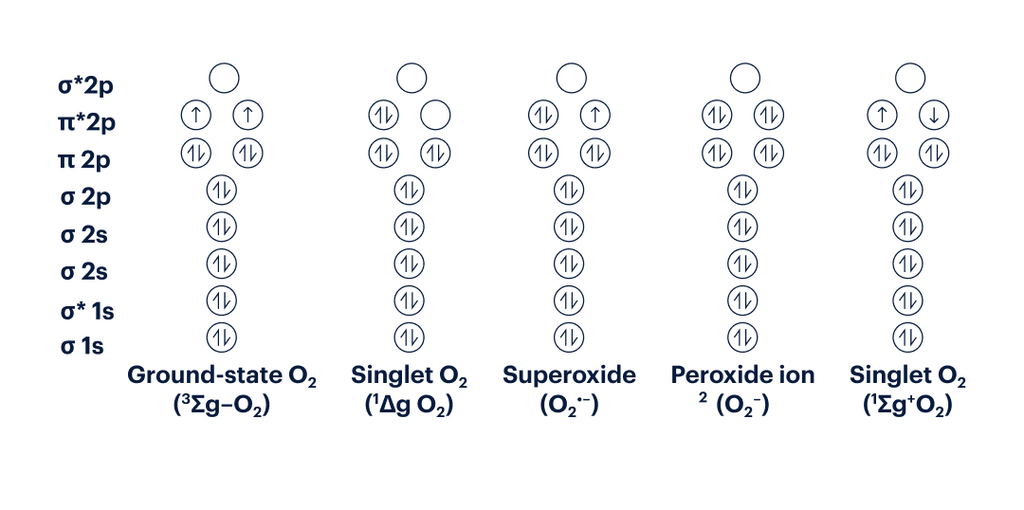
Figure 5: Electron Configuration of various ROS
Reactive Nitrogen Species (RNS)
Reactive Nitrogen Species are also formed within the body, primarily through nitric oxide (NO) reacting with other free radicals. They have been found to have a direct role in cellular signaling, vasodilation, and immune response (Drew & Leeuwenburgh, 2002). Nitric Oxide is produced within the body by enzymes called nitric oxide synthases and binds to soluble guanylate cyclase. NO itself is not very reactive but can form intermediates that have been found to affect aging. For example, NO reacts with superoxide to form peroxynitrite (ONO2-) which reacts with tyrosine to form 3-nitrotyrosine. This modification of tyrosine can also come from the nitrogen dioxide radical (NO2˙), which is formed by the reaction of hypochlorous acid (HOCl) and nitrite (NO2-).
Table 2: Radical and non radical RNS

Reactive Carbonyl Species (RCS)
Reactive Carbonyl Species are abundant in living organisms and are known to have damaging effects on various biological systems. They are formed in vivo through the oxidation of amino acids, carbohydrates, and fats and are capable of chemically modifying proteins, nucleic acids, and aminophospholipids (Semchyshyn, 2014). Other sources of RCS include industrial pollutants, cigarette smoke, and food additives. The increase of RCS within the body causes the phenomenon known as carbonyl stress, which contributes to aging and a variety of other disorders. Unsaturated RCS are much more reactive than their saturated counterparts and therefore α,β-unsaturated aldehydes, keto-aldehydes and dialdehydes produce the most biological damage (Semchyshyn, 2014).
Table 3: Biologically damaging RCS

How do Free Radicals cause damage?
Harmful effects of free radicals in the body occur when there are more reactive species present than antioxidants. Free radicals can cause damage by various mechanisms. Excited photosensitisers that come as a result of UV absorptions can induce cellular damage by two mechanisms: free radicals are produced via electron transfer and hydrogen abstraction, or the singlet oxygen free radical is formed after an energy transfer to O2 occurs. When DNA becomes oxidized it can become damaged in a variety of ways: strand breaks, sister chromatid exchange, DNA-protein crosslinks, sugar damage, abasic sites, and base modifications.
Reactive oxygen species can cause accelerated aging in the skin through collagen damage. ROS activate cell surface receptors and kinases, which increases transcription factors AP-1 and NF-kB. This increased activity of AP-1 will lead to reduced collagen synthesis due to lower production of collagens I and III. It can also cause the degradation of mature collagen by triggering the production of matrix metalloproteinases (Mitchell, 2016). This damage to existing collagen and further production can lead to accelerated aging in the skin.
UV radiation can cause damage to DNA in a direct and indirect way. The loss of epidermal stem cells (ESCs) is a large factor that leads to photoaging of the skin. ESCs play an important role in maintaining homeostasis in the epidermis and preventing photoaging. There are a variety of mechanisms that come as a result of UV-induced damage that lead to ESC depletion, including the loss of extracellular matrix integrity.

Figure 6: Pathways to UVR-induced skin aging
UV radiation directly damages DNA through the absorption of UV-A photons. This absorption leads to the formation of the singlet oxygen radical through the energy transfer from cellular photosensitizers to oxygen molecules (Panich, Sittithumcharee, Rathviboon, & Jirawatnotai, 2016). The singlet oxygen radical then produces 8-oxo-G and 8-oxo-dG via the oxidation of guanine, which leads to C-T transition mutation (Panich et al., 2016).
Free radicals can lead to DNA damage in an indirect way through the absorption of UV-B and the resulting production of Cyclobutane pyrimidine dimers. Melanin produces superoxide and nitric oxide radicals when exposed to UV which react to form peroxynitrite. Peroxynitrite degrades melanin, producing high-energy melanin derivatives that transfer their energy to DNA and produce CPDs. CPDs create linkages between adjacent pyrimidines within DNA, distorting the DNA helix. The repair of DNA though is where mutations arise that can lead to photoaging. In humans, DNA repair occurs via the nucleotide excision repair pathway (NER). There are 9 NER functioning proteins that can lead to premature skin aging when experiencing deficiencies (Panich et al., 2016).
Figure 7: Indirect damage to DNA through the production of Cyclobutane pyrimidine dimers.
What effect do free radicals have in the skin?
UV-induced photodamage is well known to contribute to skin-aging through cellular oxidative stress. The skin’s exposure to UV light can lead to acute or chronic damage dependent on the level of overexposure. Short-term overexposure will lead to damage with a quicker onset and healing process, like erythema and sunburn. The damage associated with long-term exposure to UV light and cellular oxidative stress is more severe, like photoageing and the risk of cancer. UV radiation contributes up to 80% of skin damage (Amaro-Ortiz, Yan & D'Orazio, 2014). Photo-aging can reveal itself in a variety of forms and is often associated with a droopy and leathery skin appearance.
UVB irradiation has also been shown to disrupt the skin barrier, specifically the levels of ceramide in the skin. One study found a relationship between UVB radiation and increased TEWL that coincided with a decrease in covalently bound ceramide in the stratum corneum (Takagi, Nakagawa, Kondo, Takema, & Imokawa, 2004). Interestingly, UVB irradiation did not significantly affect intracellular lipids and lead to increased levels of free ceramide and free fatty acids in the stratum corneum. Thus, this increase in TEWL, a known indicator of poor barrier function, must come primarily from the decrease in covalently bound ceramide. UVB irradiation therefore disrupts the skin barrier through the depletion of bound ceramides, but the exact mechanism is unknown.
Skin aging is a process that can occur due to both intrinsic and extrinsic effects. Intrinsic, or chronological, aging of the skin is the natural effects we see on our skin as we age. UV and environmental pollution exacerbate the natural aging process of our skin and increase the amount of damage that occurs over time. Although their effects may appear similar, the way intrinsic and extrinsic manifest in the skin differ quite a bit.
What effect do free radicals have in the skin?
UV-induced photodamage is well known to contribute to skin-aging through cellular oxidative stress. The skin’s exposure to UV light can lead to acute or chronic damage dependent on the level of overexposure. Short-term overexposure will lead to damage with a quicker onset and healing process, like erythema and sunburn. The damage associated with long-term exposure to UV light and cellular oxidative stress is more severe, like photoageing and the risk of cancer. UV radiation contributes up to 80% of skin damage (Amaro-Ortiz, Yan & D'Orazio, 2014). Photo-aging can reveal itself in a variety of forms and is often associated with a droopy and leathery skin appearance.
UVB irradiation has also been shown to disrupt the skin barrier, specifically the levels of ceramide in the skin. One study found a relationship between UVB radiation and increased TEWL that coincided with a decrease in covalently bound ceramide in the stratum corneum (Takagi, Nakagawa, Kondo, Takema, & Imokawa, 2004). Interestingly, UVB irradiation did not significantly affect intracellular lipids and lead to increased levels of free ceramide and free fatty acids in the stratum corneum. Thus, this increase in TEWL, a known indicator of poor barrier function, must come primarily from the decrease in covalently bound ceramide. UVB irradiation therefore disrupts the skin barrier through the depletion of bound ceramides, but the exact mechanism is unknown.
Skin aging is a process that can occur due to both intrinsic and extrinsic effects. Intrinsic, or chronological, aging of the skin is the natural effects we see on our skin as we age. UV and environmental pollution exacerbate the natural aging process of our skin and increase the amount of damage that occurs over time. Although their effects may appear similar, the way intrinsic and extrinsic manifest in the skin differ quite a bit.
Table 4: Intrinsic vs. Extrinsic effects on the skin’s aging process
Chronological Skin Aging |
Photoinduced Skin Aging |
Fine wrinkles |
Coarse wrinkles |
Some skin laxity |
Solar elastosis |
Unblemished skin tone |
Freckled/blotchy pigmentation |
Stratum corneum unaltered |
Thickened stratum corneum |
Epidermal atrophy |
Early stages of acanthotic epidermis End stages of atrophy |
Little cellular dysplasia |
Marked cellular dysplasia |
Modest flattening of dermoepidermal junction |
Pronounced flattening of dermoepidermal junction |
Modest reorganization of elastic fibers |
Increased deposition and massive degeneration of elastic fibers |
Modest changes in collagen bundle size and organization |
Marked changes in collagen bundle size and organization |
Reduced area of microvasculature |
Pronounced vascular abnormalities Dilation of blood vessels (telangiectasias) Increased fragility of vessels (ecchymosis) Perivascular inflammatory infiltrate |
Reduction in hair follicle number, hair thinning |
Reduction in hair follicle number and structure (hair loss and thinning) |
Reduction in number of sebaceous glands and sweat glands |
Dry skin due to reduction in number of sebaceous glands and sweat glands |
Normal melanin content |
Increased melanin content Increased number of melanocytic nevi |
Benign neoplasms (seborrheic keratoses) |
Benign neoplasia (seborrheic keratoses) Premalignant lesions (actinic keratoses) Malignant lesions (SCC, BCC) |
(Hadshiew,Eller, & Gilchrest, 2000)
What specific free radicals do antioxidants target?
Intrinsic Defense System
Antioxidants |
Target |
Mechanism |
SOD (Enzymatic) |
Superoxide (O2˙-) |
Converts superoxide radicals into H2O2 and O2 |
CAT (Enzymatic) |
H2O2 (non radical ROS) |
Contributes to reduction of H2O2 to water and O2 |
Glutathione Peroxidase (Enzymatic) |
H2O2 (non radical ROS) |
Contributes to reduction of H2O2 to water and O2 |
Non-enzymatic Antioxidants (Vitamins C & E, Glutathione, Ubiquinol) |
ROS |
Work in a coordinated manner to neutralize ROS. Ex. Glutathione restores oxidized Vitamins C & E so they can continue to scavenge ROS. |
Topical Antioxidants
Antioxidants |
Target |
Mechanism |
Vitamin C |
ROS |
Neutralizes ROS in the aqueous parts of the skin (water-soluble). Also helps in regenerating Vitamin E. |
Vitamin E - Tocopherols and Tocotrienols |
Peroxyl radical (RO2˙) |
Scavenge the peroxyl radical that would otherwise set off a chain reaction of oxidation. |
Vitamin A - Carotenoids |
Singlet Oxygen (O2) |
-carotene and lycopene scavenge singlet oxygen and inhibit lipid peroxidation. |
Vitamin A - Retinoids |
Nuclear Receptors |
Bind to RARs and RXRs, inhibiting AP-1 and MMP-1 expression and increasing collagen production and epidermal thickness. |
Epigallocatechin gallate (EGCG) |
Nitric Oxide (NO), Peroxynitrite (ONO2-), and other ROS and RNS. |
Scavenge these radicals to inhibit the transfection of NF-κB and AP-1 to downregulate the expression of inducible nitric oxide synthase (iNOS) and COX-2 enzymes. |
Dimethylmethoxy Chromanol (DMC) |
Singlet Oxygen (O2) |
Quenches singlet oxygen in SC to prevent oxidative damage. |
Resveratrol |
Peroxynitrite (ONO2-) |
Blocks oxidation of low-density lipoproteins by peroxynitrite and blocks peroxynitrite-mediated oxidation and nitration of serum and platelets proteins. |
Quercetin |
All components of antioxidant system |
Does not interact directly with any free radicals but increases or stabilizes all components of the antioxidant system. |
Silymarin |
Hypochlorous acid (HOCl), Hydroxyl Radical (HO˙), Superoxide (O2˙-), Hydrogen peroxide (H2O2) |
Scavenges ROS directly as well as inhibits specific enzymes in mitochondria involved in ROS production (NADPH oxidases and xanthine oxidases). |
Genistein |
Hydrogen peroxide (H2O2) |
Blocks peroxyl radical-mediated oxidation of LDL through hydrogen peroxide scavenging. |
Hesperidin methyl chalcone (HMC) |
Hydroxyl Radical (HO˙) |
Directly scavenges Hydroxyl radical. |
Abbreviations and Definitions
ROS Reactive oxygen species; an umbrella term that includes both radical and non radical oxygen derivatives
RNS Reactive nitrogen species; an umbrella term that includes both radical and non radical nitrogen derivatives
RCS Reactive carbonyl species; known for their damaging effects on proteins, nucleic acids, and aminophospholipids
NADPH oxidase Nicotinamide adenine dinucleotide phosphate oxidase; membrane-bound enzyme that catalyzes the production of superoxide
NADPH Nicotinamide adenine dinucleotide phosphate; the reduced form of NADP+
NADH Nicotinamide adenine dinucleotide (NAD) + hydrogen (H); an oxidative cofactor that plays a key role metabolism
NAD+ Nicotinamide adenine dinucleotide; coenzyme that plays a key role in metabolism
FAD Flavin adenine dinucleotide; a redox-active coenzyme involved in many metabolic processes
CPD Cyclobutane pyrimidine dimers; major DNA lesion that forms due to UV damage
8-oxo-G 8-oxoguanine; DNA lesion formed by ROS damage to DNA
8-oxo-dG 8-Oxo-2'-deoxyguanosine; a major product of DNA oxidation
UVR Ultraviolet radiation; part of the electromagnetic spectrum with wavelengths from 100 nm - 400 nm
UV-A Ultraviolet radiation with longer wavelengths (315-400 nm)
UV-B Ultraviolet radiation with shorter wavelengths (280-315 nm)
AP-1 Activator protein 1; a transcription factor that regulates gene expression during an immune response
NF-kB Nuclear factor kappa B; protein transcription factor that controls transcription of DNA, cytokine production and cell survival
ESC Epidermal stem cells; maintain homeostasis in the epidermis
C-T transition The most common UVA-generated mutation in the body mutation
References
Amaro-Ortiz, A., Yan, B., & D'Orazio, J. A. (2014). Ultraviolet radiation, aging and the skin: prevention of damage by topical cAMP manipulation. Molecules, 19(5), 6202-6219.
Aruoma, O. I. (1998). Free radicals, oxidative stress, and antioxidants in human health and disease. Journal of the American oil chemists' society, 75(2), 199-212.
Dizdaroglu, M., & Jaruga, P. (2012). Mechanisms of free radical-induced damage to DNA. Free radical research, 46(4), 382-419.
Domej, W., Oettl, K., & Renner, W. (2014). Oxidative stress and free radicals in COPD–implications and relevance for treatment. International journal of chronic obstructive pulmonary disease, 9, 1207.
Donohue, J. F. (2006). Ageing, smoking and oxidative stress. Thorax, 61(6), 461-462.
Drew, B., & Leeuwenburgh, C. (2002). Aging and the role of reactive nitrogen species. Annals of the New York Academy of Sciences, 959(1), 66-81.
Emerit, I. (1992). Free radicals and aging of the skin. Free radicals and aging, 328-341.
Free radicals, oxidative stress, and antioxidants in human health and disease. (1998). Journal of the American Oil Chemists’ Society., 75(2), 199–212. https://doi.org/10.1007/s11746-998-0032-9
Hadshiew, I. M., Eller, M. S., & Gilchrest, B. A. (2000). Skin aging and photoaging: the role of DNA damage and repair. American Journal of Contact Dermatitis, 11(1), 19-25.
Halliwell, B., & Gutteridge, J. M. (1985). Free radicals in biology and medicine.
Holthoff, J. H., Woodling, K. A., Doerge, D. R., Burns, S. T., Hinson, J. A., & Mayeux, P. R. (2010). Resveratrol, a dietary polyphenolic phytoalexin, is a functional scavenger of peroxynitrite. Biochemical pharmacology, 80(8), 1260-1265.
Hwang, S. W., Lee, Y. M., Aldini, G., & Yeum, K. J. (2016). Targeting reactive carbonyl species with natural sequestering agents. Molecules, 21(3), 280.
Jackson, M. (1999). Free radicals in skin and muscle: Damaging agents or signals for adaptation? Proceedings of the Nutrition Society, 58(3), 673-676. doi:10.1017/S0029665199000877
Katerina Krumova and Gonzalo Cosa, Chapter 1:Overview of Reactive Oxygen Species, in Singlet Oxygen: Applications in Biosciences and Nanosciences, Volume 1, 2016, pp. 1-21 DOI: 10.1039/9781782622208-00001
Kim E, Hwang K, Lee J, Han SY, Kim E, Park J, Cho JY. (2018). Skin Protective Effect of Epigallocatechin Gallate. Int J Mol Sci. 19(1): 173. doi: 10.3390/ijms19010173
Mailloux, R. J. (2020). An update on mitochondrial reactive oxygen species production. Antioxidants, 9(6), 472.
McMullen, R. L. (2019). Antioxidants and the skin (2nd ed.). CRC Press/Taylor & Francis Group.
Mitchell, C. L. (2016). Oxidative stress and the skin. Oxidative Stress and Antioxidant Protection: The Science of Free Radical Biology and Disease, 167-182.
Nonell, S., García‐Díaz, M., Viladot, J. L., & Delgado, R. (2013). Singlet molecular oxygen quenching by the antioxidant dimethylmethoxy chromanol in solution and in ex vivo porcine skin. International journal of cosmetic science, 35(3), 272-280.
Panich, U., Sittithumcharee, G., Rathviboon, N., & Jirawatnotai, S. (2016). Ultraviolet radiation-induced skin aging: the role of DNA damage and oxidative stress in epidermal stem cell damage mediated skin aging. Stem cells international, 2016.
Patel, R. P., McAndrew, J., Sellak, H., White, C. R., Jo, H., Freeman, B. A., & Darley-Usmar, V. M. (1999). Biological aspects of reactive nitrogen species. Biochimica et Biophysica Acta (BBA)-Bioenergetics, 1411(2-3), 385-400.
Phaniendra, A., Jestadi, D. B., & Periyasamy, L. (2015). Free radicals: properties, sources, targets, and their implication in various diseases. Indian journal of clinical biochemistry, 30(1), 11-26.
Semchyshyn, H. M. (2014). Reactive carbonyl species in vivo: generation and dual biological effects. The Scientific World Journal, 2014.
Takagi, Y., Nakagawa, H., Kondo, H., Takema, Y., & Imokawa, G. (2004). Decreased levels of covalently bound ceramide are associated with ultraviolet B-induced perturbation of the skin barrier. Journal of investigative dermatology, 123(6), 1102-1109.
Taleb, A., Ahmad, K. A., Ihsan, A. U., Qu, J., Lin, N. A., Hezam, K., ... & Qilong, D. (2018). Antioxidant effects and mechanism of silymarin in oxidative stress induced cardiovascular diseases. Biomedicine & Pharmacotherapy, 102, 689-698.
Vásquez-Garzón, V. R., Arellanes-Robledo, J., García-Román, R., Aparicio-Rautista, D. I., & Villa-Treviño, S. (2009). Inhibition of reactive oxygen species and pre-neoplastic lesions by quercetin through an antioxidant defense mechanism. Free radical research, 43(2), 128-137.
Xu, H., Zheng, Y., Liu, Q., Liu, L., Luo, F., Hu-Chen Zhou, H., Isoda, H., Ohkohchi, N., & Li, Y. (2017). Reactive Oxygen Species in Skin Repair, Regeneration, Aging, and Inflammation. In C. Filip, & E. Albu (Eds.), Reactive Oxygen Species (ROS) in Living Cells. IntechOpen. https://doi.org/10.5772/intechopen.72747